Understanding the Quantum Universe
Mysteries of Massive and [Almost] Massless Particles
Nigel Lockyer
Director, Fermi National Accelerator Laboratory
Adjunct Professor, Dept. of Physics & Astronomy
University of Pennsylvania
About the Lecture

The past two decades have seen a revolution in understanding the universe’s most fundamental particles, ushered in by several landmark discoveries. The top quark was measured to be inexplicably heavy, with a mass 300,000 times greater than the electron. The three kinds of neutrinos were found to change into one another as they propagate through space and time, something that requires them to have mass. And they were found to be surprisingly light – 100 billion times lighter than the top quark. The mystery of the wide range of particle masses was only partly resolved by the discovery of the long sought after Higgs boson at the Large Hadron Collider (LHC) in 2012. The world’s particle physicists have now embarked on an ambitious program of experiments at the LHC and at Fermilab to more completely solve the mystery. This lecture will describe the experiments and what we hope to learn from them.
About the Speaker
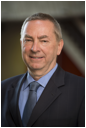
NIGEL LOCKYER began his tenure as director of Fermi National Accelerator Laboratory in September 2013. An experimental particle physicist, Lockyer spent more than two decades as a professor at the University of Pennsylvania. His research focused on high-energy particle physics using experiments at Fermilab’s Tevatron particle collider and the applications of particle physics technologies to medicine. In 2005 Lockyer became the director of TRIUMF, Canada’s national laboratory for nuclear and particle physics. Under his leadership, TRIUMF formulated a vision for ascending the world stage in nuclear physics, expanded its operations by 25 percent, developed strong partnerships among Canada’s major science laboratories and launched new international collaborations. Lockyer holds a Ph.D. in physics from The Ohio State University, is a Fellow of the American Physical Society and received the Society’s 2006 Panofsky Prize for his leading research on the bottom quark.
Minutes
President Larry Millstein called the 2332nd meeting to order on April 11, 2014 at 8:15 pm. He announced the order of business, introduced new members to the Society, and talked about the 6th meeting of the Society held in May 1871. The topics were investigations on two immense meteorites which impacted at Conception and San Gregorio, in Mexico; a paper describing examinations of tissue from an alleged hermaphrodite, and a work on alternative theories to those presented in Darwin's The Origins of Man. The minutes of the past lecture were read and approved. The President then introduced the speaker for the evening, Dr. Nigel Lockyer.
Dr. Lockyer started by a brief history of Fermilab, which was established in 1968 in Indiana with the initial goal of generating the highest-energy collisions in the world and held this record until 2008 and the inauguration of the Large Hadron Collider.
Dr. Lockyer drew the audience's attention to the puzzle of the very large range of quark masses. The top quark discovery, which Dr. Lockyer was a part of, highlights this. The top quark has the mass of 175 protons but it is still a fundamental particle with no structure that we know of. At the other extreme, the neutrino is 100 billion times lighter than a proton. There are two kinds of matter: Leptons and hadrons, the latter of which are made of quarks. Neutrinos are neutral leptons. Dr. Lockyer stated that he believes the future of particle physics lies in understanding neutrinos better.
Dr. Lockyer defined mass as the property of a body that is a measure of its inertia and is commonly taken as a measure of the amount of matter it contains and causes it to have weight in a gravitational field. Einstein demonstrated the equivalence of inertial and gravitational mass.
The current standard model features six quarks, which make up protons and neutrons. There are six leptons and four gauge bosons which regulate the strong nuclear, weak nuclear and electromagnetic forces. The Higgs boson is linked to the Higgs field. It gives mass to quarks and electrons. Dr. Lockyer pointed out that most of the proton mass comes not from the inertial mass of the quarks which make it up, but from the motions of the gluons and quarks within. The origin of the proton mass is the energy it contains. Physicists are now asking if the Higgs gives mass to the neutrinos as well.
There are three types of neutrinos: The electron, muon and tau neutrino. They were detected in the 1950s, 1960s and in 2000 at FermiLab respectively. A long-running experiment starting from the 1970s found out that fewer neutrinos from the sun were detected than was predicted by the standard solar model. Neutrino detection techniques kept improving. The missing neutrino mystery was eventually solved: Sun creates electron neutrinos, but on the way, they turn into the other two types and in fact oscillate between types as they travel. A 2001 experiment in Canada, capable of counting all three types, showed that the standard solar model prediction was correct.
For neutrinos to mix this way, they have to have mass differences. Each neutrino flavor actually has a linear combination of the mass eigenstates, m1, m2 and m3, instead of having a unique mass. In turn, each of the three masses is a mixture of the three flavors, electron, muon and tau. When a neutrino is created, it is in a pure eigenstate. As it moves through space, it oscillates between states.
Dr. Lockyer then pointed out that the electron, muon and tau neutrinos are much lighter than the electron, the muon and the tau respectively. The forces carried by the Z-boson, photon and gluons do not change the quark flavor, but the W-boson can convert quarks to one another. Quarks were experimentally observed to oscillate between states.
Dr. Lockyer then introduced the concept of charge/parity symmetry, which is the idea that physical equations should hold for particles and antiparticles alike. However, in 1964 there was a big surprise when there was a small asymmetry detected: There was a preferred direction from matter to antimatter transition for one particular process. This raised questions about the early universe; maybe different rates at that time may have caused the large difference between the matter/antimatter amounts today.
Weinberg and Salam showed that the Higgs boson gives mass to charged quarks, which make up the proton and neutron, to leptons, including the electron, and to the W and Z bosons. The quark mass is proportional to how strongly each quark couples to the Higgs field. The existence of mass and the coupling strength differences enables stable atoms. Theoretical physicists Kobayashi and Maskawa showed that a physical parameter in the CKM matrix that describes fundamental particle mixing indicates CP violation, but the violation present among the quarks is too small to explain the matter/antimatter asymmetry. We are now starting to believe that neutrinos may have much larger CP violations and may account for this asymmetry.
The detection of the Higgs boson was a great event and may lead to practical use in the coming decades, according to Dr. Lockyer. If we can understand how the high-density energy storage mechanism in mass works, maybe we can use it.
Dr. Lockyer explained that the Yukawa couplings of quarks and charged leptons to the the Higgs field gives them masses proportional to the vacuum value of the Higgs field, 174 GeV, which is the mass of the top quark; the coupling value of the top quark is 1. For electrons, the Higgs mechanism works by converting a left-handed electron to a right-handed electron. In the beginning of the universe, electrons were massless. Once the Higgs field turned on, they started oscillating between the left-handed and right-handed electrons and gained mass. This is similar for other particles. The oscillation frequency determines the particle mass, very high for the top quark and very low for neutrinos.
Dr. Lockyer then turned back to the neutrino, which holds no electrical charge, travels at nearly the speed of light, has very little mass and passes through ordinary matter with nearly no interaction. This makes them very difficult to study, even though nearly 100 billion/second of them passes through every cm2. Neutrinos created shortly after the Big Bang are still around, but have not been detected. They are naturally abundant, but they can also be created in beams to study them.
Dr. Lockyer introduced several experiments to study neutrino beams. Several tunnel experiments, hundreds of kilometers long, have detectors along the length of the tunnels as well as far detectors at the very end. These detectors weigh thousand of tons and can be warehouse-sized.
Dr. Lockyer pointed out that the matrix which describes mixing in neutrinos is very different than the same which governs quarks, which is probably significant. There are no right-handed neutrinos, so adapting the electron-mass Higgs mechanism does not quite work: A numeric factor needs to be set too small and a right-handed neutrino needs to be posited. Instead, a proposed new type of mass, called the Majorana mass, may be adapted to the Higgs mechanism, but this approach would need very high energy scale physics.
Dr. Lockyer then showed new technologies for detecting neutrinos, such as liquid argon detectors, and a new tunnel experiment, between Fermilab and South Dakota. The goals of the Long Baseline Neutrino Experiment is to quantify the muon-to-electron-neutrino conversion, to measure properties of neutrino masses, seek for clues to antisymmetry, detect supernova neutrinos and try to measure proton lifetimes.
Dr. Lockyer then mentioned the cosmic microwave background radiation, describing the fluctuations in the inflaton field. During the rapid expansion of inflation, which was faster than the speed of light, temperature stayed constant through regions that were no longer casually connected. During inflation, the quantum fluctuations in the early universe were preserved. The interaction of the gravitational field, stretched by inflation, with light created the polarization regions in the CMBR which we recently observed.
A large scale experiment with half a million detectors is planned to study inflationary expansion and gravitational field patterns on the cosmic microwave background. Since neutrinos travel at the speed of light they make gravitational potential wells more shallow, as opposed to dark matter, which accumulates and causes deeper gravitational potential waves. A fourth neutrino, a "sterile neutrino," has been proposed, which would be much heavier and add mass to the universe.
Dr. Lockyer then introduced the Dark Energy Survey, which is based on a new telescope built at Fermilab and aims to measure if the cosmological constant changes, by studying the dark energy properties of 200 million galaxies by using a 570-megapixel camera. Considering the future of particle physics worldwide, Dr. Lockyer pointed out that CERN now holds the energy frontier, and there are further plans by Europe, China and Japan. Fermilab and US are at a crossroads, and leadership as a "neutrino laboratory" is an attractive option.
After a question/answer period, Mr. President thanked the speaker, made the usual housekeeping announcements and invited guests to join the Society. At 10:13 PM, President Millstein adjourned the 2332nd meeting to the social hour.
Attendance: 76
The weather: Overcast
The temperature: 20°C
Respectfully submitted,
Zeynep Dilli
External Communications Director